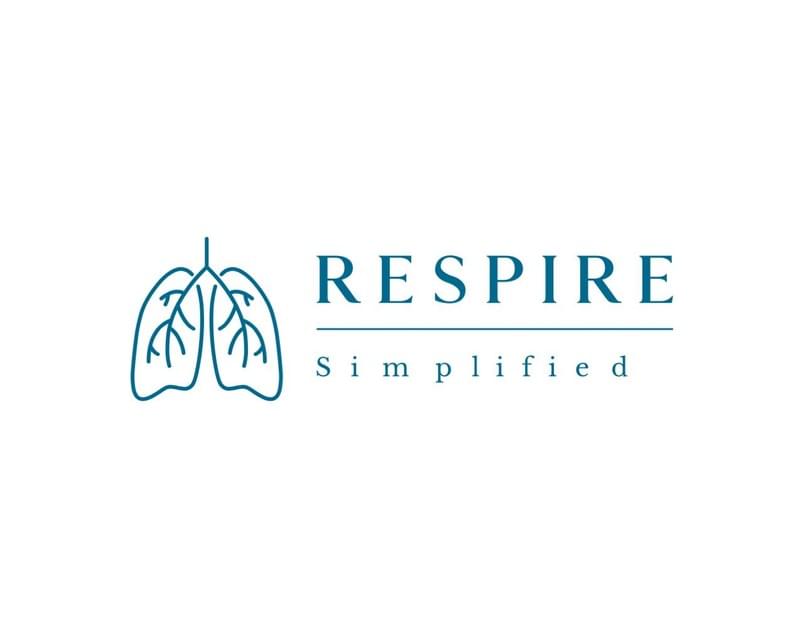
- Category
- …
- Category
- Category
- …
- Category
- FUNCTION OF RESPIRATORY SYSTEM
- RESPIRATORY MECHANICS
- VENTILATION
- PERFUSION
- VENTILATION-PERFUSION RELATIONSHIP
- DIFFUSION
- HYPOXEMIA AND HYPERCAPNIA
- TRANSPORT OF OXYGEN AND CARBON DIOXIDE IN THE BLOOD
- THE CONTROL OF BREATHING
1- FUNCTION OF RESPIRATORY SYSTEM
Functions of Respiratory System:
Each part of the resp sys has a unique function
Summarized in the image below
Breathing is the main function we will discuss in detailes!
Breathing is divided into two parts
--> Ventilation
--> Oxygenation
2- RESPIRATORY MECHANICS
Ventilation
- Defined as the repetitive movement of gas into and out of the lungs.
Into the resp sys --> Inhalation
Out of the resp sys --> Exhalation
- What is known as Respiratory Mechanics is studying the characteristics of the resp sys and the pressures allowing this breathing to happen and the interactions between them.
- Ventilation means the movement of air, and movement or motion means
Since it is a movement, then the Laws of Motion will apply to it
We will focus here on
The first law:
A body in motion remains in motion
A body at rest remains at rest
UNLESS acted upon by a force
So to create any movement, you need some kind of power, energy, force!
The third law:
For every action
There is a reaction
EQUAL in amount, OPPOSITE in direction!
So whenever movement is created, it will be faced by resistance!
In conclusion,
Ventilation will happen Only if sufficient pressure is applied to
overcome factors opposing its movement
In the resp sys, we have two factors that needs to be overcome
- Elastic Recoil
To understand it you need to understand --> Volume Pressure Relationship
-->Compliance
2. Viscous Forces
To understand it you need to understand --> Flow Pressure Relationship
--> Resistance
A. Elastic Recoil
- The elasticity of the lungs refers to their tendency to recoil to their initial size after distention>
- like the metal spring, when ever you stretch it -->it tends to go back to its initial status
- We must understand here that the initial state of the rib cage is different than the initial state of the lungs!
- If you study the rib cage alone, you will find that its initial pulling force is directed OUTward.
- The easiest way to imagine this is to think about the Jack in A Box Toy
- While the case is the opposite when you are studying the lungs alone
- You will find that its initial pulling force is directed INward
- The easiest way to imagine this is to think about a tight metal spring
- So the initial pulling force for the ribcage and the lungs are Opposite!
- And of course, we all know that in reality, the ribcage and the lungs are always functioning and interacting together
Which means those opposing forces are actually what control the balance between the lungs and the chest wall
What regulates this balance?
- Pressure-Volume Relationships
- Compliance
1. Pressure-Volume Relationships
a) The pressures:
- Although it is useful from a conceptual standpoint to discuss the lungs and chest wall as if they were isolated structures, they are, of course, linked together as a single unit.
- Nevertheless, we can still separately evaluate their elastic recoil by having a subject perform a step-wise exhalation following a maximal inspiration.
- At each point, the subject relaxes against an occluded external airway (no air flow) and both the volume change and the pressure gradient across the lungs and chest wall are measured.
Transmural pressures
- Are generated by the elastic recoil of the lungs and chest wall
- In the resp system, we have
- Transpulmonary pressure (PL)
- Gradient across the chest wall (PW)
- Each pressure is calculated by:
Pressure inside the structure – pressure outside the structure
(subtracting the pressure "outside" from the pressure "inside" each
structure)
So,
transpulmonary pressure (PL):
- Is the difference between alveolar (Palv) and pleural pressure (Ppl)
PL = Palv - Ppl
And the gradient across the chest wall (PW):
- Is equal to pleural pressure minus body surface (atmospheric) pressure (Pbs).
PW = Ppl - Pbs
Since these measurements are performed under static conditions (i.e. there is no airflow)
- Palv = the pressure measured at the airway opening (Pao) proximal to the site of airway occlusion.
- Ppl = approximated by measuring the pressure within the lower two-thirds of the esophagus (Pes) using a balloon catheter.
- Pbs = atmospheric pressure considered to be zero. (Since all pressures are referenced to it)
- Therefore:
Palv = (Pao)
Ppl = (Pes)
Pbs = zero
PL = Palv – Ppl = PL = Pao - Pes
PW = Ppl – Pbs = PW = Pes
- Note that since the lung and the chest wall are in series, the elastic recoil pressure of the entire respiratory system (PRS) at any volume is equal to the sum of the pressures generated by its two components. That is:
PRS = PL + PW
PRS = Pao – Pes + Pes
- Alternatively, the pressure-volume curve of the respiratory system can be determined directly by measuring PRS at each volume.
PRS = Palv - Pbs or
PRS = Pao
The elastic recoil pressure of the respiratory system at any volume, therefore, is simply the pressure recorded at the airway opening.
The normal sigmoid pressure-volume curve in an upright, awake, and relaxed subject.
- The pressure-volume curve of the respiratory system (PRS) is the sum of the pressures generated by the chest wall (PW) and lungs (PL) (dotted lines).
- Functional residual capacity (FRC) occurs where the sum of the 2 pressures are equal and opposite.
- Note that the chest wall contributes to much of the curvature below FRC, whereas the lung contributes most to the curvature above FRC.
- Towards the end of tidal expiration, the lungs tend to recoil inward while the chest wall tends to recoil outwards.
- These two opposing forces lead to a negative pressure within the potential space between the parietal and visceral pleurae.
- The negative intrapleural pressure (PPl) is one of the important factors that keep the patency of small airways, which lack cartilaginous support.
- The rhythmic contraction of inspiratory muscles causes cyclic changes in the dimensions of the thoracic cage and consequently comparable cyclic fluctuation of PPl.
During tidal inspiration,
- PPl drops from −5 to −8 cmH2O enforcing the intra-alveolar pressure (Palv) to drop one cmH2O below atmospheric pressure (Patm), Fig. 2a. As a result, air flows into the alveoli.
- The drop of PPl also decreases the airways resistance by dilating the small airways and thus enhancing the air flow further.
The sequence of events reverses during tidal expiration,
- When inspiratory muscles relax, dimensions of the thoracic cage decrease, PPl increases from −8 back to −5 cmH2O and Palv increases one cmH2O above Patm.
- As a result, air flows outside the alveoli following the pressure gradient, Fig. 2b.
- Tidal expiration is therefore a passive process, which needs no further muscle contraction.
During tidal breathing, whether inspiratory or expiratory,
- intra-airways (Paw) pressure is always more than PPl.
- This explains why small airways are always opened, even at the end of tidal expiration.
If inspiration above the tidal limit is required,
- accessory muscles of inspiration must be activated.
- Thoracic cage expands more leading to higher drop in PPl and Palv compared with tidal inspiration
- which explains why more air is delivered to the alveoli compared with tidal inspiration.
Alternatively, expiration below the tidal level is an active process that
- requires contraction of expiratory muscles.
- During forceful expiration, the thoracic cage is compressed to the maximum.
- Both PPl and Palv rise above Patm;
- however, Palv remains more than PPl due to the effect of elastic recoil pressure (Pel) of the alveolar wall.
As demonstrated in Fig. 2c, Paw decreases from the area next to the alveoli upwards.
- This gradual drop in Paw is secondary to simultaneous increase in the airways resistance towards the trachea.
- Taking into consideration the relatively constant PPl around the lung, each small airway can be subdivided into three segments (Fig. 2c):
- An inflated segment, where PPl is lower than Paw.
- An equal pressure point, where PPl is equal to Paw.
- An airflow limiting segment, where PPl is higher than Paw
2. Compliance
The elastic recoil of the lungs, chest wall, and respiratory system can be quantified in a number of ways.
As discussed above, pressure-volume relationships can be plotted by performing sequential measurements during step-wise exhalation.
Although this method provides a great deal of information, it is time-consuming and requires both cooperation and practice on the part of the subject.
In addition, the information obtained cannot easily be converted to numerical form.
Alternatively, a single measurement of pressure and volume can be made.
Although it is rapid and relatively easy to perform, such a measurement provides information about elastic recoil only at a specific lung volume.
A compromise between these approaches is to measure elastic recoil pressure at two volumes.
The difference between these pressures (∆P) and volumes (∆V) can then be used to calculate the compliance (C), where
C = ∆V/∆P.
Note that compliance varies inversely with elastic recoil.
That is, when compliance is low, a given pressure increment produces only a small increase in volume (i.e. the structure is stiff),
whereas
A large increase occurs when the compliance is high.
By measuring the appropriate transmural pressures during relaxation against an occluded airway, the compliance of the lungs (CL), chest wall (CW), and respiratory system (CRS) can be determined from the following equations:
CL = ∆V/∆(Pao - Pes)
CW = ∆V/∆Pes
CRS = ∆V/∆Pao
Note that compliance is simply the slope of the volume-pressure curves shown
- The compliance of the lungs, chest wall, and respiratory system is fairly constant (i.e. there is little change in slope) over a large volume range.
- Near TLC and RV, however, compliance falls (i.e. the slope decreases) as the elastic elements within the lungs and chest wall reach the limits of their dispensability.
- In the middle zone, little change in pressure results in big change in volume wide slope = high compliance.
- At TLC and RV, big change in pressure results in little change in volumes narrow slope=low compliance
What is the origin of Elastic Recoil???
Elastic recoil is produced by two factors.
1- Tissue forces
Result from the deformation of elastic elements in the lung parenchyma (i.e. elastin and collagen) and chest wall (i.e. ribs, muscles, and cartilage) and are easily understood using the analogy of a metal spring.
2- Surface forces, on the other hand,
- Results from the unique elastic properties produced by the alveoli
- Like all air-liquid interfaces, surfactant generates surface tension which tends to reduce alveolar size and increase the pressure required to maintain a given lung volume.
- According to Law of Laplace, all air-liquid interfaces (bubble) generate surface tension which tends to reduce the size and increase the pressure required to maintain a given volume.
- In this sense, alveoli are similar to soap bubbles, in which surface tension acts to both decrease volume and increase internal pressure according to the Laplace equation:
- According to this equation, alveolar pressure due to surface forces should vary inversely with alveolar size.
- If this were true,
* During inspiration -> alveolar size will increase -> elastic recoil would decrease, and
* During expiration -> alveolar size will decrease -> elastic recoil would increase
* increasing elastic recoil would lead to diffuse alveolar collapse
This, of course, does not occur.
- The solution to this problem comes from a unique property of surfactant.
* Unlike that of other liquids, the surface tension of surfactant is not constant but varies directly with the size of the air-liquid interface.
* As alveolar volume increases, surface tension rises. This more than offsets the effect of increasing alveolar radius and allows surface forces
and elastic recoil to increase with lung volume. During expiration, decreasing surface tension allows surface forces to fall, thereby decreasing
elastic recoil and preventing alveolar collapse.
B. Viscous Forces
- Under static conditions, maintaining the respiratory system at any point above or below its equilibrium volume requires only enough pressure to balance its elastic recoil.
- During the dynamic processes of inspiration and expiration, however, additional pressure must be supplied to overcome the frictional or viscous forces produced by the flow of gas through the airways.
1- Pressure-Flow Relationship
- As gas moves through the airways, pressure is required to overcome both:
* Friction at the airway surface and
* cohesive forces between gas molecules
- A pressure gradient (∆P) must, therefore, exist between the mouth and the alveoli.
- This gradient serves
- as the pressure needed to overcome viscous forces
- as the driving pressure required to produce airflow
what are the factors influencing ∆P?
1)- The most important determinant of ∆P is the caliber of the airway,
Since ∆P varies inversely with the 4th or 5th power of the airway radius (depending on whether flow is laminar or turbulent).
This means that viscous forces and the pressure required to overcome them will increase by at least 16 times when airway radius is reduced
by one-half
2)- Airway length
3)- Flow rate
4)- Density of the gas
* Is the weight per unit volume of a substance.
* For example, helium has a similar viscosity but a much lower density than oxygen or nitrogen)
5)- Viscosity of the gas
* Is a measure of the resistance of a gas or liquid to movement
* Is directly proportional to the strength of the cohesive forces between its molecules.
- Because of irregularities, protuberances, and abrupt changes in diameter, the upper airway (mouth, pharynx, and larynx) accounts for approximately 35 percent of the viscous forces during ventilation.
- The viscous forces contributed by the lower airway (below the vocal cords) occur predominantly in the first six generations.
- The small airways (less than 2 mm in diameter) account for a very small proportion of total airway viscous forces.
- Given the marked dependence of driving pressure on airway radius, it may appear counterintuitive that viscous forces should decrease as the airways become smaller.
- The key lies in the fact that as the airways divides and become smaller and smaller, their total number increases dramatically. Since the same volume of gas must pass through each generation of airways, flow through each individual airway must progressively slow until flow stops completely at the level of the alveolus. This reduction in gas velocity results in a progressive decrease in viscous forces.
Resistance
2- Resistance
soon!
2- VENTILATION
Partial Pressure of Respiratory Gases
- In a gas mixture, the pressure exerted by a gas is equal to the product of its fractional concentration (F) and the total pressure of all the gases in the mixture.
Pgas = Ptotal x Fgas
- For example, oxygen comprises about 21% of dry air (i.e. FO2 = 0.21). At sea level at a total barometric pressure (PB) of 760mmHg, the partial pressure of oxygen can be calculated as:
PO2 = PB x FO2
PO2 = 760 x 0.21 = 160 mmHg
- Dry air contains approximately 79% nitrogen (FN2 = 0.79) and only 0.04% CO2 (FCO2 = 0.0004). The partial pressures of these gases in dry air can be calculated in the same way:
PN2 600mmHg
PCO2 0.3mmHg
- When air enters the conducting airways of the lungs, it is heated and humidified.
- The partial pressure of the water vapor (PH2O) is approximately 47mmHg at body temperature.
- This means that the total pressure exerted by dry gas decreases, and the partial pressure of each gas falls. In the airways, gas partial pressure is calculated as:
PIgas = (PB – PH2O) x Fgas where PIgas is the partial pressure of inspired gas.
For example, PIO2 = (760 – 47) x 0.21 = 150mmHg.
- The partial pressure of nitrogen and carbon dioxide are calculated in the same way.
PIN2 563mmHg
PICO2 0.3mmHg
- Once gas enters the alveoli, diffusion occurs leading to a drop in the PO2 and an increase in PCO2.
- The partial pressure of gases in the alveoli cannot be measured, but they can be estimated.
- For practical purposes, we can assume that the mixed alveolar PCO2 (PACO2) is equal to the PCO2 of arterial blood (PaCO2).
- The alveolar air equation is used to calculate the average alveolar PO2 (PAO2) assuming “ideal” conditions, that is, no mismatching of ventilation and perfusion (see lecture on ventilation-perfusion relationships).
PAO2 = (PB – PH2O) FIO2 – PACO2
R
- In this equation, R is the respiratory exchange ratio.
- This is the volume of CO2 that enters the alveoli divided by the volume of O2 that diffuses into the pulmonary capillary blood over a given period of time (VCO2/VO2).
- R must also represent the ratio of CO 2 produced to O2 consumed by the tissues.
- For our purposes, R is assumed to be 0.8. That is, there is more O2 leaving the lung than CO2 entering it.
- This means that the P O2 will fall by more than the increase in PACO2.
- It will decrease by PACO2/R.
- The partial pressure of gases in the alveoli are approximately:
PAO2 100mmHg
PACO2 40mmHg
PAN2 573mmHg
- The alveolar air equation is used not only to estimate mixed alveolar PO2 but also to derive an important clinical parameter – the difference between the calculated alveolar and the measured arterial PO2.
- This value is referred to as the PA-aO2 or the A-a gradient and is normally 8-12 mmHg.
- As discussed in subsequent lectures, PA-PaO2 increases in the presence of ventilation-perfusion mismatching, shunt, and diffusion impairment.
Minute Ventilation, Alveolar Ventilation, and Anatomic Dead Space
- The tidal volume (VT) is the volume of gas that enters the lungs during a single breath.
- It is important to recognize that a significant portion of each tidal breath never reaches the alveoli or participates in gas exchange.
- This portion is named the physiologic dead space and it is divided into two spaces:
The physiologic dead space is divided into two spaces:
- Anatomic dead space:
- This is the volume of gas that fills the nose, mouth, pharynx, larynx, and conducting airways.
- Since this gas is never in contact with pulmonary capillary blood, it does not participate in gas exchange.
- As expected, the anatomic dead space volume varies with body (and airway) size.
- Although it can be measured, for practical purposes it is often estimated as 1ml per pound of ideal body weight.
2. Alveolar dead space:
- It is the portion of the tidal breath that reaches alveoli that either
--> receive no blood flow or
--> under-perfused relative to the amount of ventilation they receive
- The amount of gas entering the dead space is referred to as the dead space volume (VD).
- The alveolar volume (VA) is the volume of gas that actually reaches the alveoli AND participates in gas exchange during a tidal breath.
- It is, therefore, the difference between tidal volume and dead space volume.
VA = VT – VD
- The total volume of gas that enters or leaves the lungs each minute is referred to as the minute ventilation (VE).
- It is the product of tidal volume and respiratory rate (RR).
VE = VT x RR
- The volume of gas that enters and leaves the physiologic dead space each minute is called the dead space ventilation
VD = VD x RR
- The alveolar ventilation (VA) is the volume of gas entering and leaving the lungs each minute that participates in gas exchange:
VA = VA x RR
- It is also the difference between minute ventilation and dead space ventilation:
VA = VE – VD
The Influence of Alveolar Ventilation on PACO2
- The partial pressure of carbon dioxide in the alveolar gas (PACO2) is determined by the rates at which CO2 enters and leaves the alveoli.
- The rate at which CO2 enters the alveoli is proportional to the partial pressure of CO2 in mixed venous blood (PvCO2), which, in turn, depends on the rate of CO2 production by the tissues (VCO2).
- That is,
PACO2 α VCO2
- The rate at which CO2 is removed from the alveoli is directly proportional to alveolar ventilation.
- PACO2 is, therefore, inversely related to VA.
PACO2 α 1 / VA
- Putting these two equations together yields:
PACO2 α VCO2 / VA
or
PACO2 = K x VCO2 / VA
- Since alveolar and arterial PCO2 are essentially equal, we may rewrite this equation as:
PaCO2 = K x VCO2 / VA
- This means that if VCO2 is constant, PaCO2 will double if VA is cut in half.
- If VA is doubled, PaCO2 will be reduced by one-half.
- Since VA = VE – VD, this equation can be rewritten as:
PaCO2 = K x VCO2 / (VE - VD)
or as
PaCO2 = K x VCO2 / VE [1 – (VD / VT)]
- This final equation demonstrates the importance of the dead space to tidal volume ratio.
- As VD/VT rises, VE must increase to maintain the same PaCO2.
The Influence of Alveolar Ventilation on PAO2
- Unlike PACO2 (and PaCO2), alveolar ventilation does not directly affect PAO2.
- Instead, its effect is indirect and produced by changes in PACO2.
- By examining the alveolar air equation, it is evident that PAO2 and PACO2 must move in opposite directions. That is, if PACO2 (PaCO2) increases, PAO2 will fall.
- The opposite will occur when PCO2 decreases.
Regional Distribution of Alveolar Ventilation:
In normal subjects
- In an upright subject, Pleural pressure progressively increases (becomes less negative) between the top and bottom of the lungs (Although a single value is often used to describe the pressure in the pleural space).
- In a seated or standing subject, the volume of air reaching the alveoli increases from the apex to the base of the lungs (This can be explained by gravity-induced changes in pleural pressure)
- Since the pressure in all alveoli is zero at the end of a passive exhalation, the trans-pulmonary pressure must be higher in the upper lung zones than in the lower zones.
example 10-2=8
10-5=5
- This means that at end-expiration, alveolar volume is high at the lung apex and progressively falls in the more dependent regions of the lungs.
- During inspiration, trans-pulmonary pressure increases equally regardless of alveolar location. Since alveoli in the non-dependent regions already have a high volume, they are less compliant than alveoli in the lower lung zones.
- This means that for the same change in trans-pulmonary pressure, the amount of fresh gas entering the alveoli is greater in the dependent than in the non-dependent regions of the lungs.
4-PERFUSION
- The lungs receive blood flow from two sources: the bronchial circulation and the pulmonary circulation.
1. The bronchial arteries
- Carry oxygenated blood from the left ventricle and originate either directly from the aorta or from intercostal arteries.
- They supply oxygen to several structures in the thorax including the conducting airways, the visceral pleura, and the esophagus.
- A large proportion of the deoxygenated bronchial venous blood drains into the pulmonary veins, thereby producing an anatomic right-to-left shunt.
2. The pulmonary circulation
- Carries the mixed venous blood from all the tissues of the body and provides oxygen to the distal airways and alveoli.
- As compared with the systemic circulation, the pulmonary arteries have
* Thinner walls
* Larger lumens
* Less vascular smooth muscle (there are no muscular vessels analogous to systemic arterioles)
- These histologic features explain three important differences between the pulmonary and systemic circulations.
- First, pulmonary vascular resistance (PVR) is normally much less than (approximately one-tenth) that of the systemic circulation.
- Second, pulmonary vascular resistance is fairly evenly distributed between the arteries, capillaries, and veins, whereas arteries and arterioles account for approximately 80 percent of the systemic vascular resistance.
- Finally, the pulmonary arteries are much more distensible and compressible than their systemic counterparts.
Factors Affecting Pulmonary Vascular Resistance
Active Factors
- Pulmonary vascular resistance can be influenced by both neural and humoral factors, which alter vascular smooth muscle tone and vessel caliber.
Neural Factors
- The pulmonary circulation is supplied with both sympathetic and parasympathetic innervation.
- In general, parasympathetic stimulation causes vascular dilation and a decrease in PVR.
- While increased sympathetic activity leads to vasoconstriction and an increase in PVR.
Humoral Factors
Pulmonary vasoconstriction is caused by
- Catecholamines, (e.g. dopamine, norepinephrine, and epinephrine)
- Histamine
- PGE2, PGF2α (prostacyclin)
- Thromboxane
Pulmonary vasodilators are
- Acetylcholine
- PGE1 and PGI2 (prostacyclin)
- The most important humoral factor, however, is alveolar hypoxia.
- As PAO2 falls, vasoconstriction occurs in the pre-capillary vessels. The mechanism for this increase in vascular tone is unknown, but the effect is not mediated through the autonomic nervous system.
- Alveolar hypoxia may have a
* Direct effect on vascular smooth muscle or
* It may cause the release of other humoral mediators such as histamine, serotonin, or prostaglandins.
- Vasoconstriction may occur locally (e.g. atelectasis) or diffusely (e.g. hypoventilation, high altitude).
- Hypoxic-induced vasoconstriction is clearly a beneficial response, since it decreases blood flow to regions of the lung that are poorly ventilated.
- Unfortunately, this response is relatively weak due to the lack of vascular smooth muscle.
Passive Factors
- The distensibility, compressibility, relative lack of smooth muscle, and low intra-vascular pressure that characterize the pulmonary circulation cause PVR to be strongly influenced by a variety of extra-vascular or “passive” factors that have no effect on vascular smooth muscle tone.
Lung Volume
- When discussing the effect of lung volume on PVR, it is important to distinguish between “alveolar” and “extra-alveolar” vessels.
- Alveolar vessels, primarily the pulmonary capillaries, are affected by alveolar volume.
- During inspiration, the increase in alveolar volume causes the capillaries to be compressed and elongated, thereby increasing the resistance of these vessels.
- As lung volume falls below FRC, this process is reversed, and PVR decreases.
- The extra-alveolar vessels are exposed to pleural pressure.
- During a spontaneous inspiration, pleural pressure falls. This increases vascular transmural pressure and vessel diameter and causes vascular resistance to decrease.
- During forced expiration below FRC, pleural pressure rises and resistance increases.
- Since both the alveolar and extra-alveolar vessels contribute significantly to total resistance, a complex relationship exits between lung volume and PVR.
- As shown in Figure 1, PVR is lowest near FRC and progressively rises with both increasing and decreasing lung volume.
Cardiac Output
- In normal subjects, significant increases in cardiac output (e.g. during exercise) have very little effect on pulmonary artery pressure.
- Since intra-vascular pressure is determined by the product of resistance and flow, increases in cardiac output must be balanced by a fall in PVR.
- This decrease in vascular resistance is not mediated by alterations in vascular tone, but is instead due to two passive processes illustrated in Figure 2 - recruitment and distention.
- As flow increases, pressure rises transiently and opens or recruits capillaries and other small vessels that had been closed due to insufficient intra-vascular pressure.
- This increase in vascular pressure also causes distention of the pulmonary vasculature.
- Both of these processes lead to a fall in PVR and minimize any flow-induced increase in pulmonary vascular pressure.
Gravity
- Due to the weight of the liquid, the pressure within a column of water (or blood) is higher at the bottom than near the top.
- Similarly, intra-vascular pressure increases in the dependent (bottom) portions of the lungs.
- In an upright subject, this pressure gradient causes progressive vascular distention toward the lung bases and narrowing near the apices.
The Regional Distribution of Pulmonary Blood Flow
- Based on the above discussion, it should come as no surprise that pulmonary blood flow increases in the dependent (bottom) portions of the lungs.
- This effect is, of course, mediated by gravity-induced increases in intra-vascular pressure, which, in turn, lead to vascular distention, decreased resistance, and increased flow.
- In a seated or standing subject, blood flow is greatest at the lung bases and least at the apices.
- In a supine subject, flow increases to the anatomically dependent (dorsal) portions of the lungs.
- When a subject lies on his or her side, blood flow increases to the dependent lung.
The Zones of the Lung
- Since alveolar pressure is fairly uniform, and intra-vascular pressure varies due to the effect of gravity,
- alveolar pressure may exceed vascular pressure in the non-dependent (top) regions of the lungs. Based on the relationship between pulmonary arterial (Pa), pulmonary venous (Pv), and alveolar (PA) pressures, Dr. John West described three lung zones (Figure 3).
- In Zone 1, PA > Pa > Pv.
* Here, no blood flow occurs because the alveolar vessels are completely collapsed.
- In Zone 2, Pa > PA > Pv.
* Here, blood flow occurs, but the pressure gradient driving that flow is Pa – PA, not Pa –
Pv.
- In Zone 3, Pa > Pv > PA.
* Here, blood flow is driven by the difference between pulmonary arterial and venous pressure.
- The extent of each of these zones is dependent
1. On the magnitude of pulmonary vascular and alveolar pressures.
* For example, low intra-vascular pressures (e.g. from hypovolemia) and high alveolar pressure (e.g. forced
expiration, positive pressure ventilation) will create larger Zone 1 and 2 regions.
* On the other hand, low alveolar pressure and/or high intra-vascular pressure will increase Zone 3 regions.
2. Body position also determines the extent and location of the lung zones.
* For example, in an upright subject, Zone 1 (usually minimal or absent) is at the apex, Zone 2 is in the mid-
lung, and Zone 3 is at the base.
* In a supine subject, Zone 1 (if present) will be in the ventral portion of the lungs while Zone 3 will be in the
dorsal regions.
Fluid Flow Across the Pulmonary Capillaries
- Like all capillaries, the junctions between the endothelial cells of the pulmonary capillaries are permeable to fluids.
- The net movement of fluid across the pulmonary capillaries is described by the Starling equation:
Qf = Kf [(Pc – Pi) – σ(πp – πi)]
where Qf = net fluid flow;
Kf = filtration coefficient (proportional to capillary permeability);
Pc = capillary hydrostatic pressure; Pi = hydrostatic pressure in the lung interstitium;
σ = reflection coefficient (describes the ability of the capillary to retain solute);
πp = colloid osmotic pressure of the plasma;
πi = colloid osmotic pressure of the lung interstitium.
These factors are illustrated in Figure 4
- Normally, there is a small amount of net fluid flow from the capillaries to the pulmonary interstitium.
- This fluid drains into the pulmonary lymphatics and is removed from the lung.
- Under pathological conditions, pulmonary lymphatic drainage can increase as much as tenfold.
Based on the above discussion, interstitial and alveolar pulmonary edema can potentially result from
- Increased capillary hydrostatic pressure
- Increased capillary permeability (increased Kf and/or decreased σ)
- Impaired lymphatic drainage
- Decreased interstitial hydrostatic pressure
- Decreased plasma oncotic pressure
- Increased interstitial oncotic pressure
Clinically, the first two factors are by far the most important.
تم إنشاء هذا الموقع باستخدام Strikingly